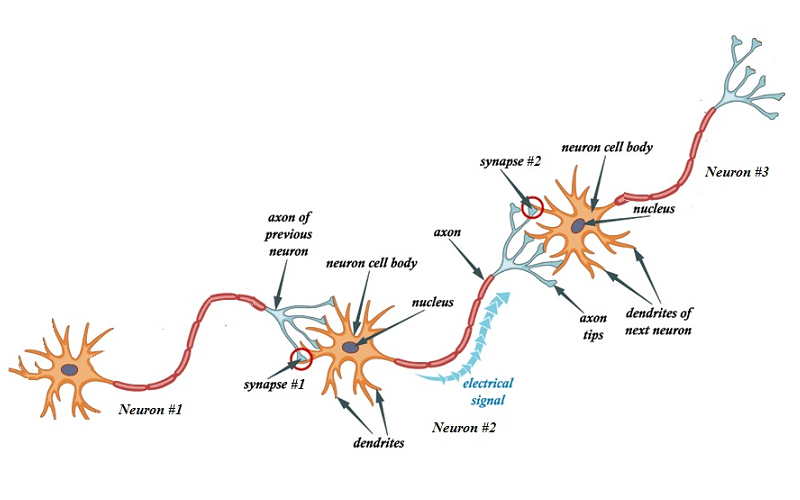
In the intricate labyrinth of the human brain, a myriad of processes occur simultaneously, orchestrating our thoughts, memories, and behaviors. One such process, often overshadowed by the well-known action potential, is electrotonic conduction. Imagine a whisper that travels in the hushed corridors of the nervous system, silently but crucially shaping the orchestra of neuronal communication. Electrotonic conduction is that whisper. A subtle, passive form of signal propagation, this phenomenon plays a pivotal role in the vast symphony of our brain’s activities.
Contents
Introduction to Electronic Conduction and Neuronal Communication
The complexity of the human brain has always been a subject of profound fascination. Comprising a staggering number of neurons intricately connected in a vast network, the brain’s means of communication is both intricate and efficient. At the heart of this communication system is the transmission of electrical signals between neurons.
While most of us are familiar with the concept of action potentials or the ‘electrical spikes’ that travel along the length of a neuron, there’s another, less talked about but equally crucial, mode of signal propagation: electrotonic conduction.
Explanation of Neuronal Communication
Neurons, often called the “building blocks” of the nervous system, have the remarkable ability to transmit information via electrical and chemical signals. Each neuron is adept at receiving, processing, and sending out information. They accomplish this by generating electrical changes within their cell membranes.
The most famous of these is the action potential—a rapid, all-or-nothing electrical event that propagates along the neuron’s length. But not all electrical changes lead to these dramatic spikes. Before an action potential is triggered, there’s a silent, passive form of communication that takes place, which sets the stage for many of the neuron’s decisions.
Brief Overview of Electrotonic Conduction and its Importance
Electrotonic conduction refers to the passive spread of voltage changes along the neuron, without the generation of a full-blown action potential. Think of it as a stone dropped in a pond: the ripples generated spread outwards, but as they move further from the source, they decrease in amplitude.
Similarly, in electrotonic conduction, the signal attenuates or diminishes as it travels. This might make it sound less impactful compared to the robust action potential, but electrotonic conduction serves its unique purpose. It helps in integrating signals from multiple sources, determining the likelihood of an action potential, and modulating the overall responsiveness of the neuron.
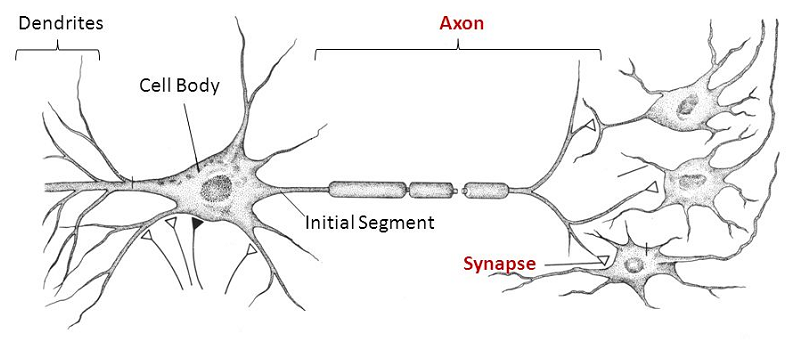
Neurons: The Basic Building Blocks
In our journey to understand electrotonic conduction, it’s pivotal to first familiarize ourselves with the core components of the nervous system: the neurons. These remarkable cells have evolved over millions of years, adapting to effectively transmit and process the vast amount of information required to coordinate even the simplest of our actions. As the foundational units of the brain and the entire nervous system, they form intricate circuits that underlie our every thought, feeling, and behavior.
Anatomy of a Neuron
Neurons are not just any cells; they possess a unique structure tailored for their specialized functions. Every part of the neuron is strategically designed to facilitate the efficient flow of information [1].
Cell Body (Soma)
The soma, or the cell body, is the central hub of the neuron. It contains the nucleus, which houses the genetic material of the cell, and is responsible for maintaining the health and functionality of the neuron. The soma integrates signals received from other neurons and determines whether the neuron should generate an action potential or not. In a way, it’s the neuron’s decision-making center.
Dendrites
Branching out from the soma like the roots of a tree, dendrites are the primary receivers of information in a neuron. They have a tree-like structure, providing a vast surface area to collect signals from neighboring neurons. The more branches or dendrites a neuron has, the more input it can receive. These inputs are usually in the form of neurotransmitters, chemicals released by other neurons, which can generate electrical changes in the dendrite’s membrane. It’s these electrical changes that may travel passively as electrotonic potentials towards the soma.
Axon
The axon is a long, slender projection that emerges from the soma. It acts as the neuron’s transmitting unit. Once the cell body decides to send a message, it generates an action potential, which then travels along the axon’s length to its end. Here, at the synaptic terminals, it prompts the release of neurotransmitters into the synaptic cleft, a tiny space between neurons, thereby passing the message to the next neuron or target tissue. While our focus, electrotonic conduction, typically occurs in the dendrites and soma, understanding the axon is crucial for appreciating the entire signal propagation process in a neuron.
Importance in Brain Function and Cognitive Processes
The architecture of a neuron is not just about its individual function but also about its collaborative role in the vast neuronal network. When neurons connect, they form pathways that underpin every cognitive and motor function of our bodies. From the simple act of touching a hot surface and reflexively pulling our hand away, to complex cognitive tasks like problem-solving or creating art, neurons are at play.
Electrotonic conduction, while silent and passive, greatly influences the outcome of these neuronal interactions. By modulating the integration of various signals at the dendrites and soma, it shapes the response of a neuron. This passive spread of voltage can determine whether an action potential will be initiated or not, thus influencing the outcome of various brain functions [2].
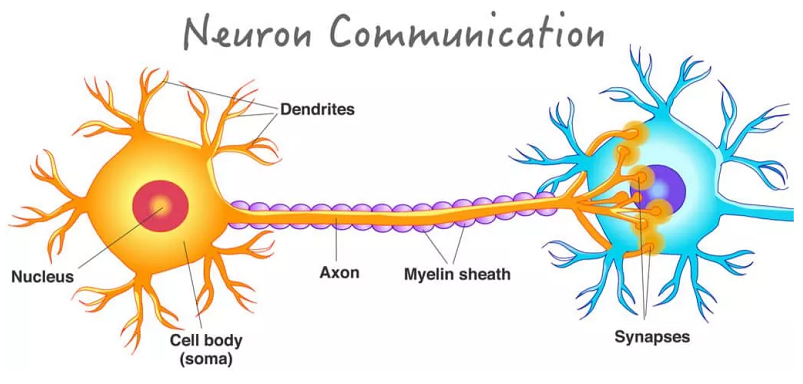
Understanding Electrotonic Conduction
Now that we’ve covered the intricacies of a neuron’s anatomy and its collaborative role in brain functions, we can shift our focus to the heart of our exploration: electrotonic conduction. While action potentials often steal the spotlight in discussions of neuronal signaling, electrotonic conduction plays its quiet yet influential part in shaping the communication landscape of our brains.
Definition and Key Concepts
Electrotonic conduction refers to the passive spread of electrical disturbances through the neuron. Unlike action potentials, which are actively propagated with consistent amplitude across the neuron, electrotonic potentials decay over distance. Imagine it as a wave of energy dissipating as it moves further from its source. This passive spread arises due to the inherent electrical properties of the neuronal membrane and doesn’t rely on the active mechanisms that drive action potentials.
How it Differs from Action Potentials
The distinction between electrotonic conduction and action potentials is critical to fully grasp the diverse modes of neuronal signaling. They are the yin and yang of neuronal communication, each with its unique features and roles.
Lack of All-or-Nothing Response
An action potential operates on an all-or-nothing principle. When a specific threshold is reached, the neuron fires a signal of a consistent magnitude. If it doesn’t reach this threshold, no action potential is generated. In contrast, electrotonic conduction doesn’t work on this principle. The magnitude of the electrotonic potential is proportional to the strength of the initial stimulus. So, stronger stimuli lead to larger passive potentials and vice versa [3].
Decremental Nature of the Signal
Electrotonic potentials diminish in amplitude as they travel across the neuron. This decremental nature is in stark contrast to action potentials, which remain uniform in size as they propagate. Think of electrotonic conduction like the fading sound of a whisper as you move further away from the source, while action potentials are akin to a shout that remains equally loud regardless of your distance from the person shouting.
The Role of Passive Properties in Electrotonic Conduction
For electrotonic conduction to occur, certain passive properties of the neuron come into play. These properties are intrinsic to the neuron’s membrane and interior, determining how the neuron responds to incoming signals.
Membrane Resistance (Rm)
This refers to how resistive the neuron’s membrane is to the flow of electrical current. A high membrane resistance means that fewer ions can pass through, allowing the electrotonic potential to spread further. Conversely, a low resistance causes the potential to decay more rapidly.
Axoplasmic Resistance (Ri)
The axoplasm, or the internal fluid of the neuron, also offers resistance to the passage of electrical signals. A higher axoplasmic resistance causes the electrotonic potentials to decrease more rapidly as they travel.
Membrane Capacitance (Cm)
Capacitance refers to the neuron membrane’s ability to store electrical charge. When the membrane has high capacitance, it can hold more charge, causing the electrotonic potentials to spread more slowly.
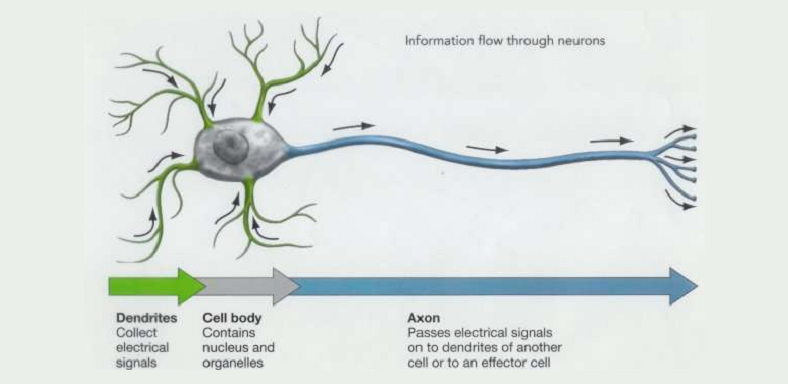
The Biophysics Behind Electrotonic Conduction
To truly appreciate the role of electrotonic conduction in shaping neuronal communication, we must understand the underlying principles that govern its behavior. Biophysical attributes, derived from the interplay of biology and physics, set the stage for this silent traveler.
Cable Theory and its Relevance
One of the primary biophysical frameworks that helps us understand electrotonic conduction is the Cable Theory. Rooted in the principles of electrical circuits, Cable Theory treats neurons, especially their dendrites and axons, as cylindrical cables. By doing so, it provides valuable insights into how electrical signals, both active and passive, spread across the neuron.
In Cable Theory, the neuron’s passive electrical properties—like membrane resistance, axoplasmic resistance, and membrane capacitance—are considered in conjunction with the neuron’s geometry to predict the behavior of electrotonic potentials. Understanding the neuron through this lens allows us to visualize why and how electrotonic signals decay over distance and why they can influence a neuron’s responsiveness.
Length Constant (Lambda) and Time Constant (Tau)
Two fundamental parameters arise from the Cable Theory that significantly influence electrotonic conduction: the length constant (lambda) and the time constant (tau). These constants help describe and quantify the behavior of passive potentials within the neuron.
Understanding the Significance of Lambda
Lambda, or the length constant, is a measure of how far an electrotonic potential travels before its amplitude decreases to about 37% of its original value. It’s determined by the relationship between membrane resistance (Rm) and axoplasmic resistance (Ri). Specifically, lambda increases with increasing membrane resistance and decreasing axoplasmic resistance [4].
The greater the value of lambda, the further an electrotonic potential can spread without significant decay. This means that in neurons with a higher length constant, passive potentials can influence regions farther from the site of origin, playing a more extensive role in the cell’s integrative functions.
How Tau Influences Signal Propagation
Tau, the time constant, deals with the temporal aspect of electrotonic conduction. It defines the time it takes for the voltage of an electrotonic potential to reach about 63% of its maximum value after a sudden change. This constant is influenced mainly by membrane resistance (Rm) and membrane capacitance (Cm).
A longer time constant (greater tau) means the neuron responds more slowly to a change in membrane potential. This can have implications for how quickly a neuron integrates incoming signals and makes decisions about firing action potentials.
Advantages and Limitations of Electrotonic Conduction
While electrotonic conduction plays a silent yet crucial role in shaping neuronal communication, it does not operate in isolation. Like every biological process, it comes with its own set of advantages and limitations, intricately balanced to optimize neural function.
The Advantages of Electrotonic Conduction
Electrotonic conduction, with its quiet nature, offers several benefits that are paramount to the efficient functioning of the neuron.
Rapid Signal Propagation
One of the key strengths of electrotonic conduction is its speed. Since it’s a passive process that doesn’t rely on the active opening and closing of ion channels, like action potentials do, signals spread almost instantaneously. This swift transmission can be crucial, especially when neurons need to integrate inputs rapidly.
Integration of Multiple Inputs
Given that neurons often receive signals from multiple sources simultaneously, electrotonic conduction plays a critical role in integrating these various inputs. The dendrites and soma can accumulate these passive potentials, and depending on their collective strength and timing, decide whether or not to fire an action potential.
Modulation of Neuronal Responsiveness
Electrotonic potentials, by adjusting the membrane potential closer to or further from the threshold for action potential generation, can modulate a neuron’s responsiveness to incoming signals. This can allow neurons to fine-tune their reactions based on the context of the incoming signals.
Limitations of Electrotonic Conduction
While electrotonic conduction provides various benefits, it also has inherent limitations that shape its role within the neuron.
Decremental Spread of Signal
The most significant limitation of electrotonic conduction is the decremental nature of the signal. As the signal travels, it weakens, meaning that distant parts of the neuron may not be as strongly influenced by the electrotonic potential as regions closer to the site of origin.
Limited Range of Influence
Because of the decremental spread, electrotonic conduction has a limited range of influence. While it’s effective in localized regions, for long-distance communication, like from the sensory receptors in our toes to our spinal cord, action potentials are the preferred mode of transmission.
Dependence on Passive Properties
The effectiveness of electrotonic conduction is largely determined by the neuron’s passive properties, such as membrane resistance and axoplasmic resistance. This means that any changes in these properties, perhaps due to damage or disease, can significantly affect electrotonic conduction and, by extension, neuronal communication [5].
Role of Electrotonic Conduction in Brain Functions
How does the silent mode of signaling contribute to the rich tapestry of brain activity and cognition? As it turns out, the understated role of electrotonic conduction offers key contributions to several vital brain functions.
Influence on Neural Integration
At the heart of neural processing lies the task of integration: the neuron’s ability to collect, combine, and make sense of myriad signals it receives.
Temporal Summation
When a neuron receives frequent inputs from a single source in a short span of time, these signals can accumulate, thanks to electrotonic conduction. This phenomenon, known as temporal summation, can increase the chances of the neuron reaching the threshold to fire an action potential. In this way, rapid but individually weak inputs can collectively have a significant influence on the neuron’s response.
Spatial Summation
Similarly, if a neuron receives simultaneous inputs from multiple sources, these can be added together or “summed” spatially. Electrotonic conduction allows these multiple, simultaneous passive potentials to combine, possibly driving the neuron closer to the threshold of an action potential.
Modulation of Synaptic Strength
Electrotonic conduction can play a role in modulating the strength of synapses, the connections between neurons.
Amplifying Weak Signals
A weak synaptic input might not by itself induce a strong enough post-synaptic potential to influence the neuron’s decision-making. However, when combined with other signals via electrotonic conduction, its influence can be amplified, making it a contributor to the overall activity of the neuron.
Dampening Overwhelming Inputs
Conversely, when a neuron receives an overwhelmingly strong input, the spread of electrotonic potentials can distribute this charge, potentially preventing the neuron from being excessively activated. This can serve as a protective mechanism, ensuring that neurons do not become overexcited.
Influencing Learning and Memory
The brain’s ability to adapt and restructure its connections, known as plasticity, is fundamental to learning and memory. Electrotonic conduction might subtly influence this process.
Adjusting Neuronal Sensitivity
As previously discussed, electrotonic potentials can move the membrane potential closer to or further from the action potential threshold. This adjustment can influence how a neuron responds to future inputs, possibly playing a role in synaptic plasticity, a cornerstone of learning and memory.
Facilitating Coincidence Detection
For certain learning processes, it’s crucial for a neuron to detect the coincidental arrival of two separate signals. The passive spread of electrotonic potentials can aid in this “coincidence detection,” ensuring that the neuron effectively recognizes and responds to simultaneous inputs.
References
[1] Electronic Properties of Axons and Dendrites
[2] Solitonic conduction of electrotonic signals in neuronal branchlets
[3] Neurons | Organismal Biology
[4] Electrical Signaling in Neurons
[5] Neural Communication

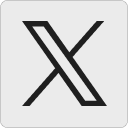





